Nuclear Fusion – a little bit of context
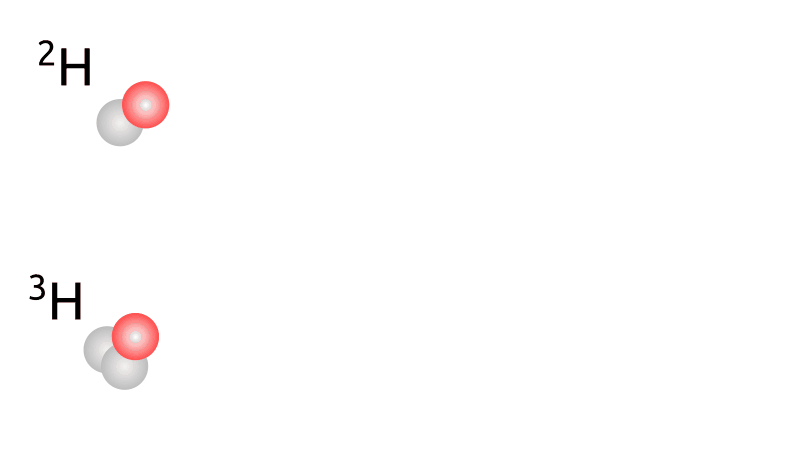
The deuterium-tritium fusion reaction. Red are protons, grey are neutrons. © Dominik Freinberger
My last blog post was devoted to giving an introduction on what molecular dynamics, the method I am working with this summer, is. In this post I want to talk more about the other side of the coin, the area of application of my project: Nuclear fusion and fusion reactors.
The Fusion Process
Nuclear fusion is the atomic reaction that makes our sun and the stars shine. It happens when two (or more) atomic nuclei come close enough together for the strong nuclear force to overcome the Coloumb repulsion between the protons in the nuclei. For certain (light enough) elements, this reaction is accompanied by a release of energy, as the fusion product is of lower potential energy than the individual nuclei as a whole. It is the difference in binding energy of the nuclei before and after fusion that is released. According to Einstein’s mass-energy equivalence E=mc2 the release of energy is equivalent to a mass defect, i.e. a loss of mass of the final nucleus compared to the individual initial nuclei.
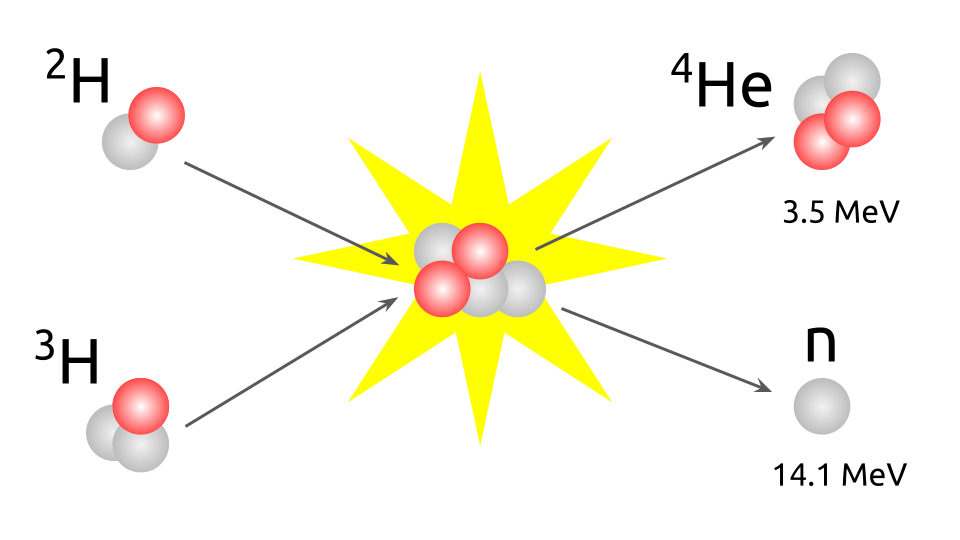
In our sun hydrogen nuclei 1H fuse into a helium core 4He in a multi-stage process known as the proton-proton chain. The most promising fusion partner candidates on earth are the hydrogen isotopes deuterium 2H and tritium 3H by means of their fusion reaction rate relative to energy input in terms of temperature. Deuterium-tritium fusion produces a helium nucleus and a free neutron, accompanied by 17.6 MeV of kinetic energy of the resulting particles. With 14 MeV, the neutron carries the lion’s share of the energy, which has to be converted into practically usable energy.
While millions and millions of tons of hydrogen fuse into helium every single second in the sun, releasing an enormous amount of energy as a result, this process is not so simple to replicate on Earth. In the Sun and other stars, besides the high temperatures and high density, it is the enormous gravitational pressure that brings the atomic nuclei close enough together for a long enough period of time to overcome (actually tunnel through) the Coloumb barrier and allow the nuclear forces to take over and enable nuclear fusion to occur.
Since it is not possible to realize such pressures on Earth, the fusion fuel must be brought to even higher temperatures than in the core of the Sun – tens to hundreds of millions of degrees Kelvin. At these temperatures, electrons and atomic nuclei are separated from each other and form an electrically conducting plasma. Additionally, the plasma must be confined as in the Sun to resist expansion due to it’s own pressure. Since no material on Earth can withstand these temperatures and the plasma would cool down immediately if it touched any wall material, the plasma must be controlled in a very special way. Arguably the most well known and best researched approach to this is magnetic confinement as implemented in a Tokamak or Stellarator reactor. Both concepts exploit the fact that charged particles in a magnetic field experience Lorentz force and follow helical paths along the field lines. In both designs, the plasma is kept floating in a vacuum using strong external magnetic fields. As my project deals with studying properties of parts of the first reactor concept, the Tokamak, I will discuss this one in more detail.
The Tokamak Reactor
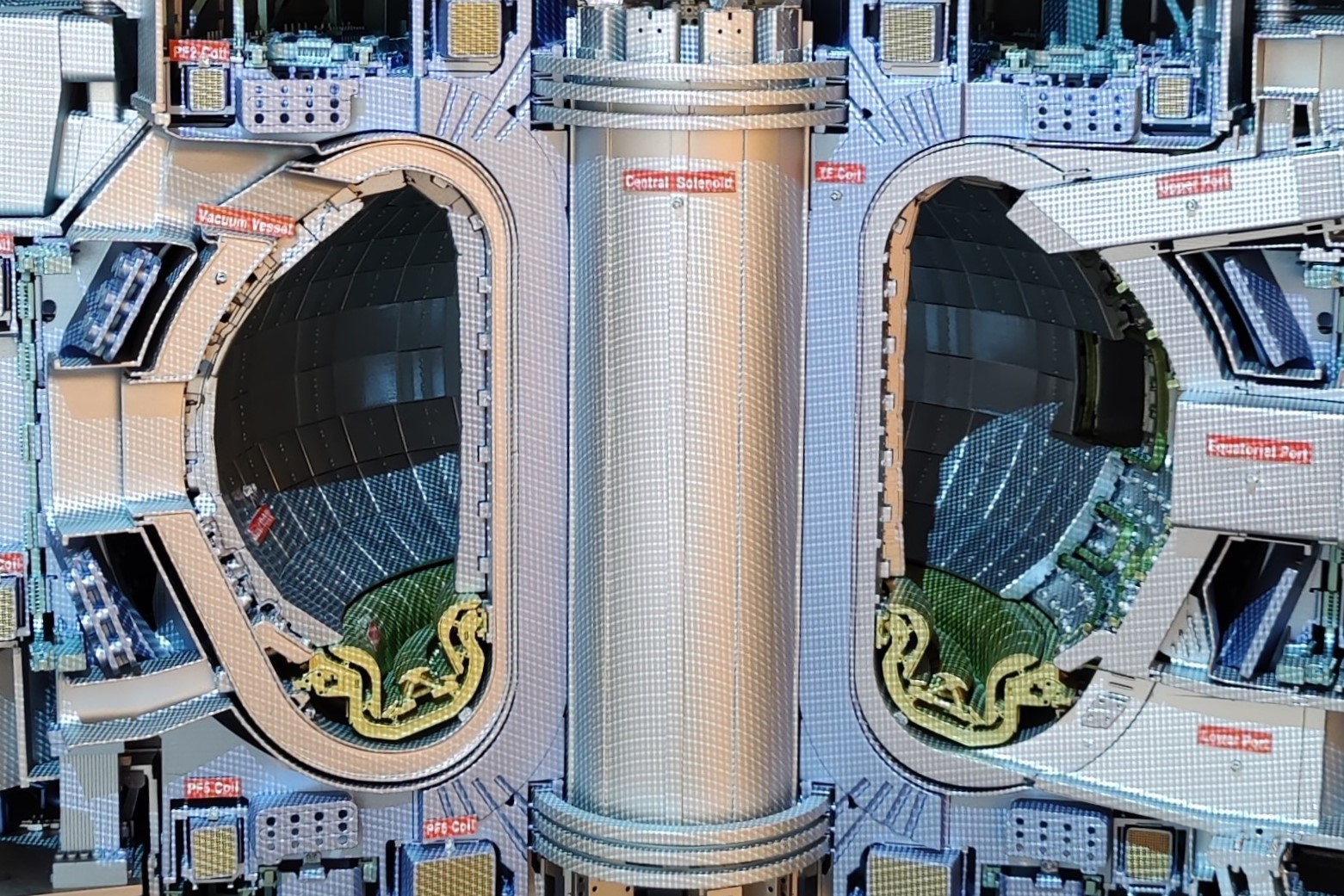
The term Tokamak originates from the Russian word токамак which is an abbreviation for toroidal’naya kamera s magnitnymi katushkami – meaning toroidal chamber with magnetic coils.
The name says it all: The Tokamak is composed of a toroidal chamber (doughnut shape) which is surrounded by torodial magnetic field coils. These magnets provide for the confinement of the plasma. In addition, a central magnet, the solenoid, induces a strong electric current in the plasma itself, which serves to stabilize the plasma. This principle can be compared to a transformer where the plasma acts as the secondary winding. Another set of poloidal field coils is used for positioning and shaping the plasma.
An important component of future tokamak reactors is the divertor. It is located in the lower part of the plasma chamber and is used to remove “fusion ash” – helium, unburned deuterium and tritium as well as impurities – from the fusion plasma. Parts of the divertor are so-called plasma facing components and are therefore exposed to particularly high radiation loads. One candidate material for this application is tungsten, which I am investigating in the course of my project to determine how its thermal conductivity is affected by irradiation.
ITER
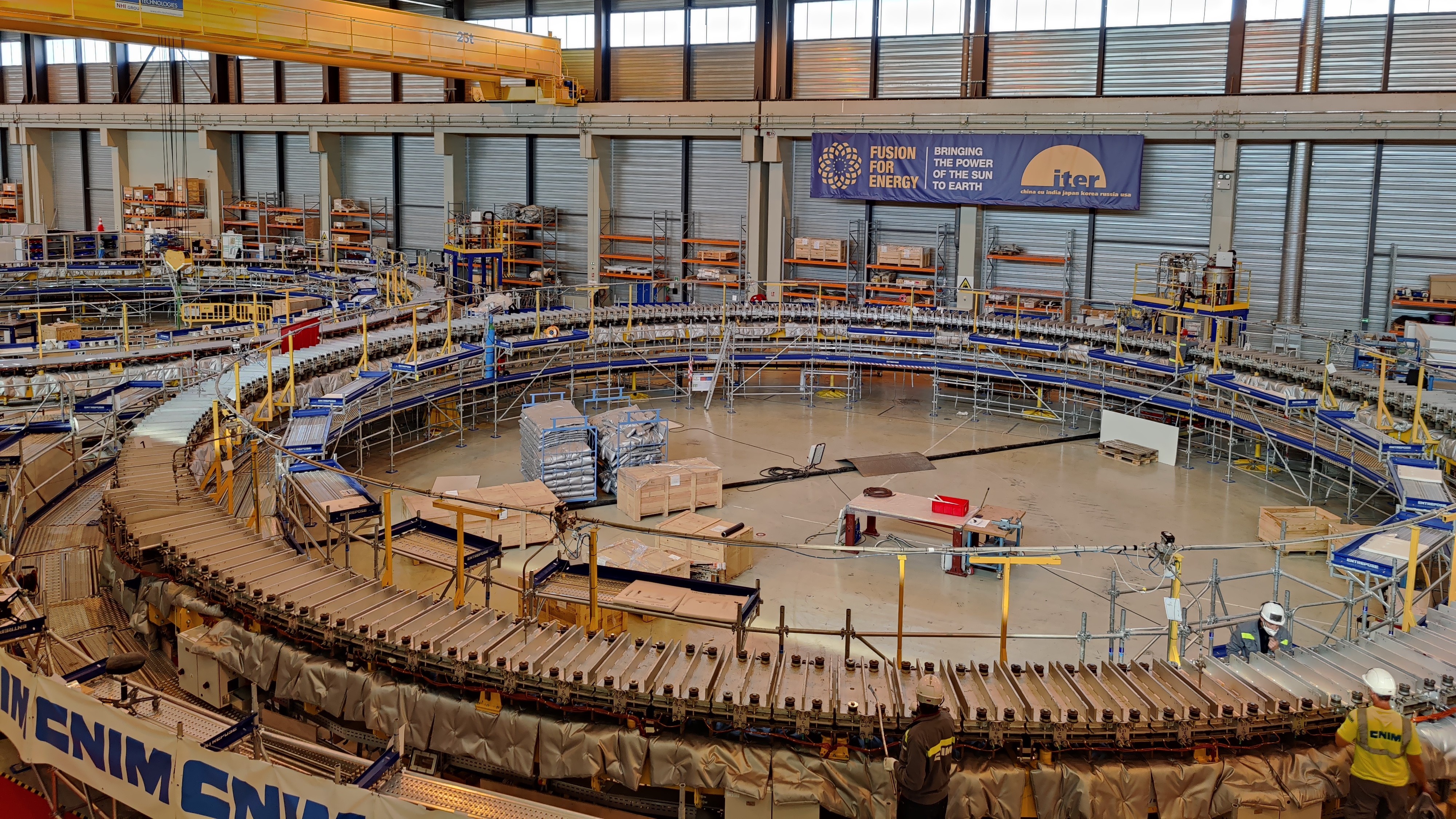
Finally, I would like to mention a particularly impressive example of a tokamak: ITER – International Thermonuclear Experimental Reactor. Currently under construction, this massive collossus of a fusion reactor is being built in the south of France, at the Cadarache nuclear research center. In a few years, the first plasma is to be ignited here, ultimately proving the feasibility and economic viability of nuclear fusion. ITER is an unprecedented international collaboration on a global scale.
In September 2021, I had the unique opportunity to participate in a guided tour to the ITER site. The picture above shows the on-site construction of the aforementioned poloidal field coils – to give an idea of the scale of this project.
Just as the first plasma will soon be ignited in ITER, I hope this post was able to spark some interest in nuclear fusion.